



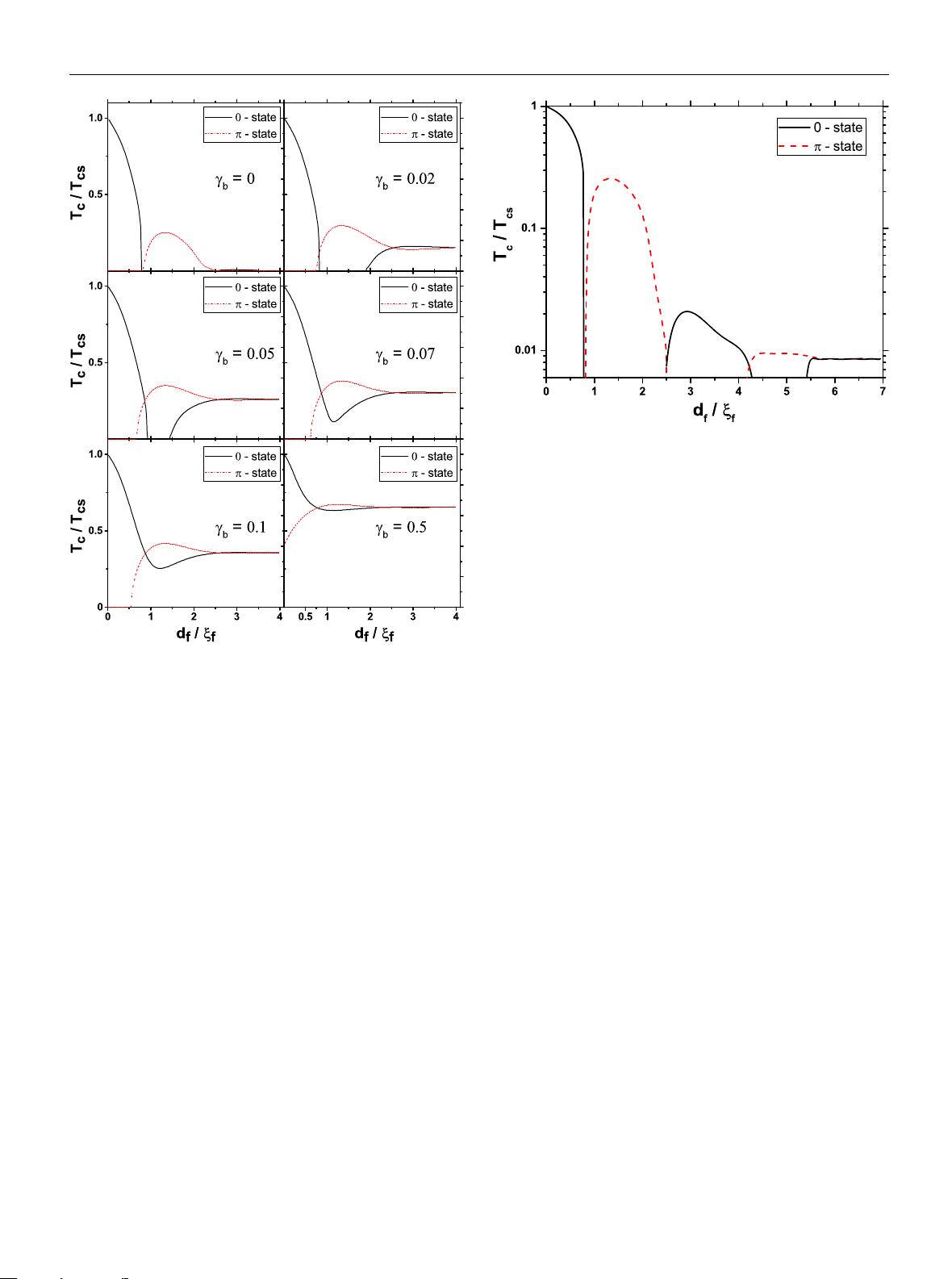
read more
One of the interesting problems would be to extend the MMA to the nonequilibrium case by using the KeldyshUsadel Green ’ s function approach [ 84 ]. It is also interesting to study more complex phases in S/F multilayers in the MMA, extending the results of Ref. [ 63 ] obtained in the SMA.
In S/F/S trilayers, only the state with highest Tc is realized at certain d f , i.e., when increasing d f the dashed red line appears above the solid black line, the 0-π transition occurs, and the structure switches to the π phase state.
With decrease of the S-layer thickness ds in S/F/S trilayers, the critical temperature is suppressed due to the inverse proximity effect, which becomes more profound in the case104502-6of small ds.
For fully transparent S/F interfaces, γb = 0, the critical temperature appears at d f ∼ ξh (we note that in their case ξh = 0.54ξ f , since h = 6.8πTcs), reaches a maximum at a particular d f , and with further increase in d f eventually drops to zero.
Other possible extensions will include spin-orbit coupling effects in equilibrium [72] and nonequilibrium cases [90] and considering Tc in S/F/S junctions in the presence of an equilibrium supercurrent [91].
In this case, the critical temperature Tc vanishes when the π phase state becomes energetically unfavorable in a certain interval of d f , and at d f ξ f the Tc eventually tends to zero.
2s πTcs d2Fs dx2− |ωn|Fs + = 0. (1) In the F layer (−d f /2 < x < d f /2), the Usadel equation can be written asξ 2f πTcs d2Ff dx2− (|ωn| + ih sgn ωn)Ff = 0.
(13) According to the Usadel Eqs. (1)–(3), there is a symmetry relation F (−ωn) = F ∗(ωn), which implies that F+ is a real while F− is a purely imaginary function.
At the borders of the S layers with a vacuum, the authors naturally havedFs(±ds ± d f /2) dx= 0. (6) The solution of the Usadel equation in the F layer depends on the phase state of the structure.
The self-consistency Eq. (14) and boundary conditions Eqs. (15), together with the Usadel equation for F+s ,ξ 2s πTcs d2F+s dx2− ωnF+s + 2 = 0, (17) will be used for finding the critical temperature of the S/F/S structure both in 0 and π phase states.
This situation corresponds to an S/F/S structure enclosed in a ring, where the π phase shift can be fixed by applying the magnetic flux quantum for any d f .
Thus the authors confirm the importance of using the MMA in a wide range of parameters in the case of S/F/S trilayers, where 0-π phase transitions are possible.
Using the boundary condition Eq. (10) the authors arrive at the effective boundary conditions for F+s at the boundaries of the right S layer,ξs dF+s (d f /2)dx = W 0,π (ωn)F+s (d f /2), (15a)dF+s (ds + d f /2) dx= 0, (15b) where the authors used the notationsW 0,π (ωn) = γ As( γb + Re B0,πf ) + γ As∣∣γb + B0,πf ∣∣2 + γ (γb + Re B0,πf ) , As = ksξs tanh(ksds), ks = 1ξs√ ωnπTcs .
To provide complete behavior of the critical temperature in S/F/S trilayers, the authors calculate Tc(d f ) dependencies in both 0 and π phase states by using the MMA and show them on the same plot, see Fig.