
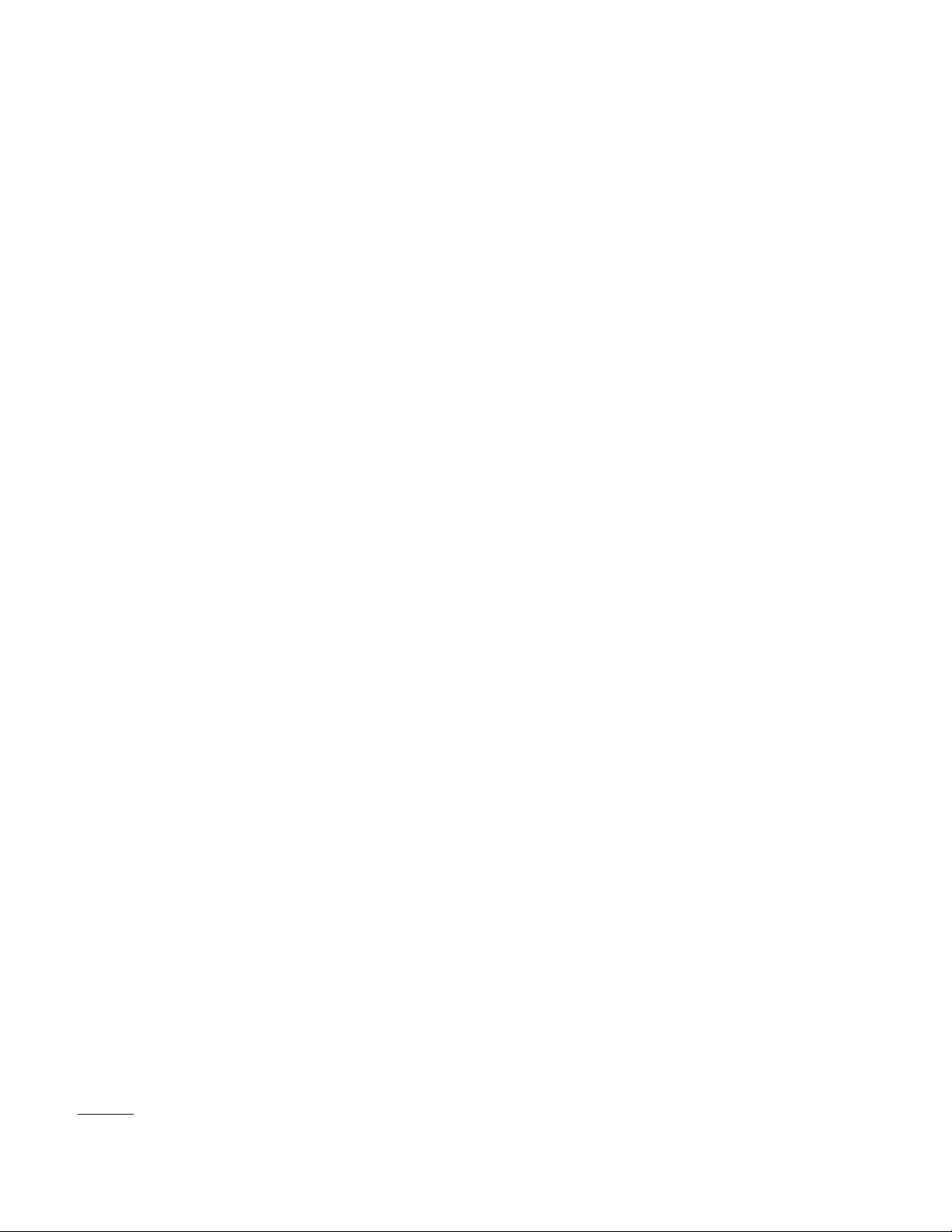

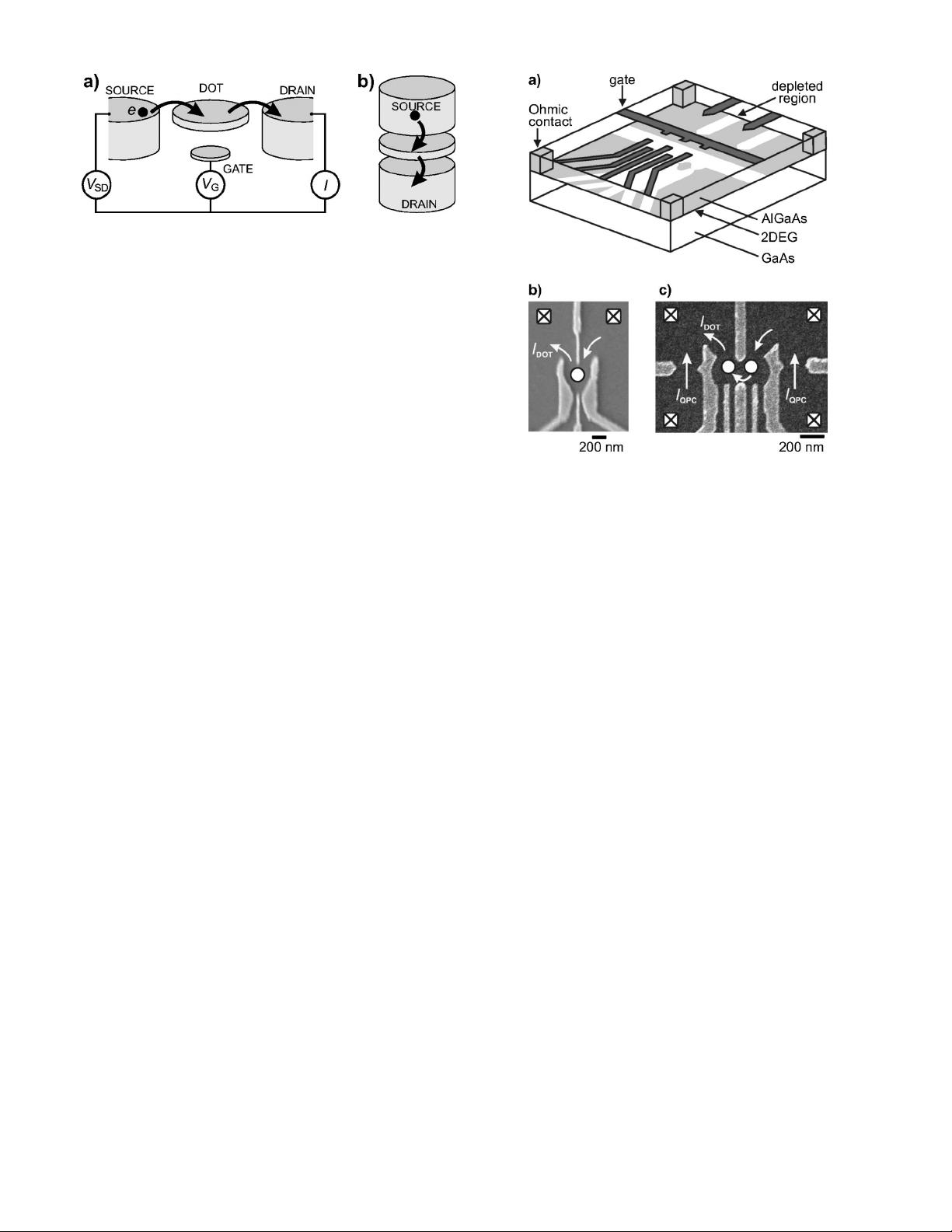

read more
Spin filling can also be deduced from excited-state spectroscopy without changing the magnetic field Cobden et al., 1998 , provided the Zeeman energy splitting EZ=2 EZ =g BB between spin-up and spin-down electrons can be resolved.
due to the effect of the Zeeman splitting, the matrix element in Eq. 22 picks up another factor of EZ assuming only single-phonon processes are relevant .
Since both nuclear spins and the localized electron spin are quantum objects, the hyperfine coupling could in principle create entanglement between them if both the electron spin and nuclear spins had a sufficiently pure initial state; see Braunstein et al., 1999 .
Electric fields affect spins only indirectly, so generally spin states are only weakly influenced by their electric environment as well.
In fact, the nuclear field has been the main limitation on the fidelity of spin rotations in recent electron-spin resonance experiments in a quantum dot see Sec. IX.
by starting from zero electrons and thus zero spin and tracking the change in spin at subsequent electron transitions, the total spin of the ground state can be determined Willems van Beveren et al., 2005 .
If the effect of the nuclear field on the electron-spin coherence could be suppressed, the spin-orbit interaction would limit T2, to a value of 2T1 to first order in the spin-orbit interaction , which, is as the authors have seen, a very long time.
The phonon-induced transition rate between the renormalized states n , l , ↑ 1 and n , l , ↓ 1 is given by Fermi’s golden rule an analogous expression can be derived for relaxation from triplet to singlet states, or between other spin states := 2n,l1 nl↑
Sz of the ground state changes by more than 12 , which can occur due to many-body interactions in the dot, can lead to a spin blockade of the current Weinmann et al., 1995; Korkusiński et al., 2004 .
The probability that a triplet state is formed is given by 3 T / S+3 T , where the factor of 3 is due to the degeneracy of the triplets.
The signal-to-noise ratio is enhanced significantly by lock-in detection of GQPC at the pulse frequency, thus measuring the average change in GQPC when a voltage pulse is applied Sprinzak et al., 2002 .
If the tunnel rates for spin up and spin down are not equal, the amplitude of the current can be used to determine the spin filling.
The authors now show that the same result follows from the quantum-mechanical description, where the spin-orbit coupling can be treated as a small perturbation to the discrete orbital energy-level spectrum in the quantum dot.
the authors note that the full quantum description is required to analyze correlations between microscopic nuclear spin states and the single electron spin state, as, e.g., in a study of the entanglement between electron and nuclear spins.
In GaAs, estimates for vary from 103 to 3 103 m/s, and it follows that the spin-orbit length lSO = / m* is 1–10 m, in agreement with experimentally measured values Zumbühl et al., 2002 .
The error probabilities are found to be =0.15 and =0.04, where is the probability that a measurement on the state S T yields the wrong outcome T S .
The sensitivity of the charge sensor to changes in the dot charge can be optimized using an appropriate gate design Zhang et al., 2004 .